【Research Background】
In order to meet the demands of practical energy storage applications, it is necessary to develop secondary batteries with high energy/power density and long charge-discharge life. Due to its extremely high theoretical specific capacity (3860 mAh g–1) and low redox potential (−3.04 V vs. standard hydrogen electrode), metallic lithium is considered to be an ideal lithium anode material. However, from the perspective of battery practical application, the metal lithium negative electrode needs to be significantly improved in terms of rate, specific capacity and charge and discharge stability. On the one hand, the surface capacity of the lithium negative electrode is required to be greater than 10 mAh cm-2 in order to achieve the energy density of the battery to reach 500 Wh kg-1; on the other hand, the next generation of battery applications requires the lithium negative electrode to have a current greater than 10 mA cm-2 Achieve fast charge and discharge at low density. However, lithium metal is prone to uncontrollable dendrite growth, electrode volume expansion, and instability of the solid electrolyte interface membrane during the charge-discharge cycle, resulting in safety and cycle problems that are limited. These problems will be more serious under the conditions of high current density and large capacity charging and discharging, which will greatly affect the cycle stability and service life of the battery.
【Job Introduction】
In response to this, the research group of Professor Liang Jiajie of Nankai University used the method of polymer covalent cross-linking to improve the mechanical strength and toughness of the three-dimensional framework based on MXene for the first time to prepare a metal lithium carrier with excellent conductivity, lithium affinity, and mechanical stability. Prepare lithium anode material by depositing lithium metal. Specifically, MXene nanosheets and polysiloxane undergo a silanization reaction to form a covalently cross-linked MXene/silver nanowire three-dimensional framework. Compared with the control sample (non-crosslinked MXene framework), the covalently cross-linked MXene framework shows excellent mechanical strength and toughness, which is beneficial to alleviate the huge internal stress changes during the rapid and deep lithium deposition/stripping process Impact, to ensure the structural integrity of the three-dimensional framework carrier during long-term charge and discharge cycles. The lithium metal negative electrode in a symmetrical battery has a stable charge and discharge cycle of more than 3000 cycles (3000 hours) at a capacity of 10 mAh cm-2 and a current density of 20 mA cm-2. The article was published in the international journal Advanced Functional Materials. Graduate students Qian Xiaojuan and Fan Xiangqian are the first authors of this article.
【Content Expression】
The presence of lithium-philic functional groups (-O, -F, -OH) on the surface makes 2D MXene (Ti3C2Tx) nanosheets favorable for lithium deposition. Through hydrolysis, polycondensation and silylization reactions, MXene nanosheets are cross-linked with polysiloxane, which greatly enhances the mechanical stability of the MXene skeleton. AgNW is further integrated with the cross-linked MXene framework to improve the conductivity and lithium affinity of the framework. Covalently cross-linked MXene/silver nanowire (AgNW) (C-MXene/AgNW) as a lithium composite anode exhibits ultra-high capacity and excellent long cycle life.
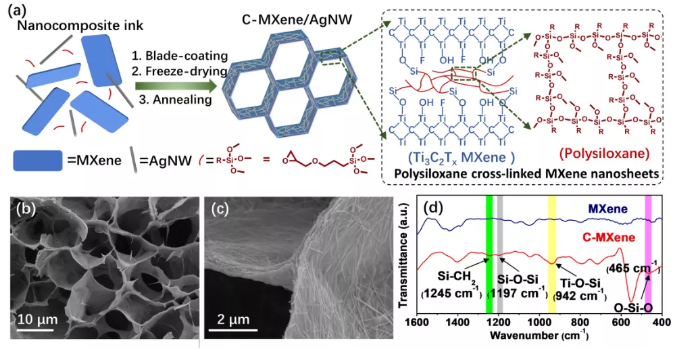
Figure 1 (a) Schematic diagram of the preparation of C-MXene/AgNW framework; (b) SEM cross-sectional view of C0.5-MXene/AgNW framework; (c) SEM enlarged at the junction of C0.5-MXene/AgNW framework; (d) Infrared spectra of pure MXene and C-MXene/AgNW framework.
Figure 1 is a schematic diagram of the synthesis of the C-MXene/AgNW framework. As a silane coupling agent, 3-glycidoxypropyltrimethoxysilane is widely used to improve the mechanical properties of polymer composites and can be hydrolyzed and polymerized into polysilicon Oxane. AgNW was chosen because of its good electrical conductivity and good lithium affinity, which can promote the rapid and uniform deposition of lithium. The hydrogel prepared by the suction filtration method is scraped and dried to obtain a three-dimensional porous framework. In order to promote the formation of crosslinks between MXene and polysiloxane through Si-O-Ti bonds, the multidimensional C-MXene/AgNW framework was annealed at 100°C for 4 h. The best mass ratio of MXene, AgNW and silane in the C-MXene/AgNW framework is 1:2:0.5, which is defined as C0.5-MXene/AgNW in the article. For comparison, the mass ratios of MXene, AgNW and silane of 1:2:0.2 and 1:2:0.7 are defined as C0.2-MXene/AgNW and C0.7-MXene/AgNW, respectively, without the definition of adding silane coupling agent It is MXene/AgNW.
Figures 1b and 1c show the multidimensional structure of the C0.5-MXene/AgNW framework, with a continuous AgNW network on the MXene framework. Its average macropore size is about 10 μm, and its mass density is about 0.1548 g cm−3, which helps to limit the lithium deposited in the porous framework. The SEM of Figure 1c shows that the C0.5-MXene/AgNW framework is multilayered with a thickness of 150 nm. In addition, there are no cracks, creases or boundaries around the wall joints, indicating the structural integrity of the covalently cross-linked framework. The new characteristic peak Ti-O-Si in the Fourier infrared spectrum of Figure 1d at 942 cm-1 confirmed the cross-linking of MXene nanosheets with polysilane. The three new characteristic peaks at 1245, 1197 and 465 cm-1 are attributable to the stretching vibration of Si-CH2, Si-O-Si and the bending vibration of O-Si-O. These results indicate that the hydrolysis, condensation, and silicification of 3-glycidoxypropyltrimethoxysilane are responsible for the crosslinking of MXene nanosheets and polysiloxane.
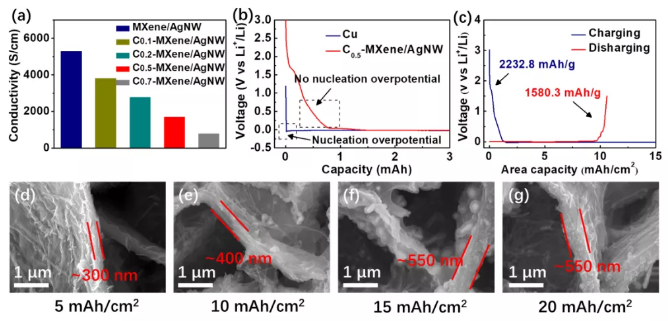
Figure 2 (a) The conductivity of pure MXene/AgNW and C-MXene/AgNW framework with different silane coupling agent addition; (b) the conductivity of 0.5mA cm-2 on Cu and C0.5-MXene/AgNW framework Voltage curve of current density deposition of lithium (c) Depositing 15 mAh cm-2 of lithium at a current density of 0.5mA cm-2 on the framework of Cu and C0.5-MXene/AgNW, and the voltage curve of stripping lithium to 1.5V; different deposition Schematic diagram of the enlarged C0.5-MXene/AgNW framework after the amount of lithium (d) 5 mAh cm-2; (e) 10 mAh cm-2; (f) 15 mAh cm-2; (g) 20 mAh cm-2.
The combination of AgNW and MXene can significantly increase the conductivity of the framework and reduce the overpotential of lithium nucleation. Figure 2a shows that although the conductivity of C-MXene/AgNW decreases as the mass ratio of insulating polysiloxane increases, the conductivity of C0.5-MXene/AgNW is still as high as 1693 S cm−1, which helps the skeleton The charge inside is transferred quickly. Figure 2b shows that when lithium is deposited on the C-MXene/AgNW framework to prepare a lithium composite anode (Li@C-MXene/AgNW), since the nucleation overpotential of lithium on silver is zero, metallic lithium is first deposited on AgNW. Then evenly distributed on the skeleton wall. Electron micrographs of electroplating different capacities of lithium on the C0.5-MXene/AgNW framework are shown in Figure 2d-g. The wall thickness of C0.5-MXene/AgNW has increased from 150nm (Figure 1c) to about 300 nm (Figure 1c). 2d) (deposition of 5 mAh cm-2); after depositing 15 mAh cm-2 of lithium, the wall thickness of the framework increases to about 550 nm (Figure 2f). After depositing 20 mAh cm−2 lithium, the wall thickness of the skeleton still maintains about 550 nm (Figure 2g). When 15 mAh cm-2 lithium is deposited on the C0.5-MXene/AgNW framework, it is equivalent to depositing 2232.8 mAh g-1. When the lithium is stripped to 1.5V at a current density of 0.5 mA cm-2, the electrodes The reversible specific capacity is about 1580.3 mAh g-1 (above 10 mAh cm-2, calculated based on the mass of the entire electrode), which is equivalent to a lithium utilization rate of 70.8%.
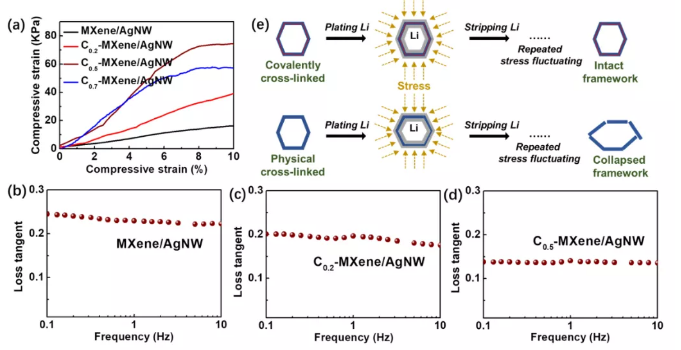
Figure 3 (a) Stress curve of MXene/AgNW, C0.2-MXene/AgNW, C0.5-MXene/AgNW, C0.7-MXene/AgNW framework; when the oscillation change is 0.5%, the relationship between mechanical loss angle and frequency Schematic diagram (b) MXene/AgNW; (c) C0.2-MXene/AgNW; (d) C0.5-MXene/AgNW; (e) Schematic diagram based on MXene framework structure changes during repeated lithium deposition/stripping process.
Figure 3b-d shows that when tested by dynamic mechanical analysis (DMA), when the oscillation strain is 0.5%, the loss factor of C0.5-MXene/AgNW is the smallest. For C0.5-MXene/AgNW, in the frequency range of 0.1 to 10, the loss factor remains unchanged at approximately 0.13, indicating that the C0.5-MXene/AgNW framework has good elasticity. On the contrary, the loss factors of MXene/AgNW and C0.2-MXene/AgNW frameworks with low crosslinking density both decrease with increasing frequency, indicating that their structures are unstable. The C0.5-MXene/AgNW framework formed by the combination of high Young modulus and low loss factor has small structural changes and good structural elasticity. Figure 3e compares the structural stability of physically cross-linked MXene/AgNW foam and covalently cross-linked C0.5-MXene/AgNW foam during repeated lithium deposition/stripping processes. C0.5-MXene/AgNW withstood stress fluctuations and maintained a complete frame after lithiation and delithiation. In contrast, the framework of MXene/AgNW foam cannot withstand the stress caused by lithium deposition, resulting in shape changes and final structure collapse after repeated deposition/stripping.
Figure 4 (a) Comparison of symmetrical battery long-cycle performance under 20 mA cm-2 current density and 10 mAh cm-2 capacity (Li@MXene/AgNW is green, Li@C0.2-MXene/AgNW is blue, Li@C0.5-MXene/AgNW is red); (b) 30 mA cm-2 current density, 10 mAh cm-2 capacity, symmetric battery long cycle performance comparison chart (Li@C0.2-MXene/AgNW is Blue, Li@C0.5-MXene/AgNW is red); (c) Li@C0.5-MXene/AgNW anode and the high current density reported in the literature, the number of cycles, current density, Comparison diagram of capacity (d) The rate performance of Li@C0.5-MXene/AgNW anode at current density of 1, 5, 10, 20, 30, 40 mA cm-2, and the time for depositing/stripping lithium is per circle 10 min.
Figure 4a shows that when the current density is 20 mA cm-2 and the plating/stripping time is 0.5 h (corresponding to an areal capacity of 10 mAh cm-2), the Li@C0.5-MXene / AgNW negative electrode shows an ultra-low value of 15 mV Overpotential for 250 h (corresponding to 250 electroplating/stripping cycles). In sharp contrast, Li@C0.2-MXene/AgNW (less covalent crosslinking) showed a higher and more unstable overpotential during 180 hours of deposition/stripping, and then the overpotential was cycled 200 Sudden increase after hours. Li @ MXene/AgNW (without covalent crosslinking) has a stabilization time of no more than 25 hours. What impressive is that the Li@C0.5-MXene/AgNW composite negative electrode can work stably for 3000 hours (3000 laps of electroplating/electroplating/) under high current density (20 mA cm-2) and high surface capacity (10 mAh cm-2). Peel). It maintains an overpotential as low as 50 mV in both charging and discharging conditions. When the current density is further increased to 30mA cm-2 and the areal capacity is 10 mAh cm-2, the symmetrical battery assembled with the Li@C0.5-MXene/AgNW composite negative electrode can be cyclically stripped stably for 400 hours without any signs of short circuit . In contrast, batteries assembled with Li@C0.2-MXene/AgNW composite negative electrodes can only be electroplated/stripped a few times (Figure 4b). The huge difference in the cycle stability of symmetric batteries shows the advantages of C0.5-MXene/AgNW framework lithium in terms of rapid and deep electroplating and peeling, which reflects excellent mechanical stability and toughness.
Figure 4c shows that the Li @ C0.5-MXene/AgNW anode is cycled for 3000 h under such high current density (20 mA cm-2) and surface capacity (10 mAh cm-2) conditions, and its long-term cycle stability is better than All lithium composite anodes previously reported. The rate performance of Li@C0.5-MXene/AgNW composite negative electrode was evaluated by assembling symmetrical batteries at different current densities and cycling for 10 minutes per cycle. As shown in Figure 4d, at current densities of 1, 5, 10, 20, 30, and 40 mA cm-2, the corresponding area capacities are 0.17, 0.83, 1.7, 3.3, 5.0, and 6.7 mAh cm-2, and beyond The potentials are approximately 12, 28, 36, 52, 60 and 66 mV. Having such a low overpotential at ultra-high current density indicates that the ion transport speed is fast and the interface is stable during the cycle.
Figure 5 Cross-sectional topography (a) C0.5-MXene/AgNW in the original state; (b) C0.5-MXene/AgNW under the conditions of 20 mA cm-2, 10 mAh cm-2 lithium deposition/exfoliation 100 cycles After; (c) MXene/AgNW under the conditions of 20 mA cm-2, 10 mAh cm-2 after lithium deposition/stripping for 100 cycles; the COMSOL model of the pressure distribution caused by lithium deposition on different frameworks (d) C0. 5-MXene/AgNW; (e) MXene/AgNW.
Figure 5a shows that the thickness of the C0.5-MXene/AgNW framework is about 300 μm, and shows a complete porous framework structure before cycling. After 100 cycles of electroplating/peeling 10 mAh cm-2 at a current density of 20 mA cm-2 (the last step is electroplating), C0.5-MXene/AgNW maintains its thickness (≈300μm) and the integrity of the porous structure Sex (Figure 5b). This indicates that the covalently cross-linked framework has excellent mechanical stability, and can fully adapt to changes in lithium volume and withstand huge stress fluctuations during lithium deposition/stripping. In contrast, under the same cycling conditions, after 100 electroplating/stripping cycles, the MXene/AgNW framework without covalent crosslinking completely collapsed (Figure 5c). In order to further compare the structural stability of C0.5-MXene/AgNW and MXene/AgNW frameworks, COMSOL M is used The ultiphysics software analyzed their mechanical properties to describe the stress distribution of materials during lithium deposition (Figure 5d, 5e). Although the force distribution of the two frameworks is similar after depositing the same amount of lithium, the structural deformation of the MXene/AgNW framework with poor mechanical stability (Figure 5e) is much greater than that of the strong and stable C0.5-MXene/AgNW Skeleton (Figure 5d). This makes the non-crosslinked MXene/AgNW framework more prone to structural collapse during cycling
Figure 6 (a) Comparison of long-cycle performance of pure Li-Cu||NCM and Li@C0.5-MXene/AgNW||NCM full battery at 10C rate over 1000 cycles; (b) Li-Cu|| The rate performance of NCM and Li@C0.5-MXene/AgNW||NCM full battery ranges from 1C to 20C; the detailed voltage curve diagram of rate performance test (c) 1C; (d) 5C; (e) 10C.
In order to determine the potential of Li@C0.5-MXene/AgNW framework in practical battery applications, Li@C0.5-MXene/AgNW composite negative electrode (plated with 15 mAh cm-2 lithium) and commercial positive electrode LiNi0.5Co0. 2Mn0.3O2 (NCM523) is assembled into a full battery. As shown in Figure 6a, the Li @ C0.5-MXene/AgNW||NCM full battery exhibits extremely stable cycle performance. The capacity remains as high as 60 mAh g-1 after 1000 cycles at 10C rate, and the average capacity decay per cycle is only 0.043%, and the coulombic efficiency is stable at 98.7%. On the contrary, the capacity of Li-Cu||NCM full battery decreases rapidly, and finally decays to less than 10 mAh g-1 only after 100 charge/discharge cycles. In addition, Li @ C0.5-MXene/AgNW||NCM full battery exhibits better rate performance than Li-Cu||NCM full battery, especially at high rates (Figure 5b). Li@C0.5-MXene/AgNW||NCM full battery can maintain discharge capacity up to 138, 128 and 107 mAh g-1 at current densities of 1 C, 5 C and 10 C (Figure 6c–e). In contrast, Li-Cu||NCM full batteries only provide discharge capacities of 130, 95 and 49 mAh g-1 at 1C, 5C and 10C, showing poor cycle stability.
【in conclusion】
The strategy of this work focuses on optimizing the mechanical stability of the lithium metal framework, thereby significantly improving the cycle life of the lithium metal anode. The conductive and lithophilic MXene/AgNW framework formed by the combination of weak van der Waals forces will collapse due to the inability to withstand huge stress changes during the lithium deposition/stripping cycle. On the contrary, this work introduces covalent crosslinking through the silicification reaction with polysiloxane, which significantly improves the mechanical strength and toughness of the skeleton, which is beneficial to keep the structure of the skeleton intact during the long cycle. Therefore, the lithium metal composite anode prepared in this work exhibits excellent reversibility under ultra-high current density and ultra-high areal capacity, making the practical application of high-performance lithium metal batteries possible.
Xiangqian Fan, Yang Yang, Xinlei Shi, Yang Liu, Hongpeng Li, Jiajie Liang , and Yongsheng Chen, A MXene-Based Hierarchical Design Enabling Highly Efficient and Stable Solar-Water Desalination with Good Salt Resistance, Adv. Funct. Mater., 2020, DOI:10.1002/adfm.202007110
About the Author
Liang Jiajie, professor and doctoral supervisor. In 2011, he graduated from the Polymer Research Institute of Nankai University (supervisor: Professor Yongsheng Chen), and then joined the research group of Professor Qibing Pei (Pei Qibing) of the University of California, Los Angeles, as a postdoctoral researcher and assistant R&D engineer. Joined the School of Materials Science and Engineering of Nankai University in 2016. The main research direction is Printing functional composite materials and application research in the construction and integration of wearable functional device. In recent years, he has published more than 30 papers as the first and corresponding author, including Nature Photonics, Nature Communications, Advanced Materials, Nano Letters, ACS Nano, Advanced Energy Materials, Advanced Functional Materials and other internationally renowned journals and magazines, of which 8 were selected ESI Top 1% highly cited papers. The paper has been cited more than 6000 times. In 2016, he was selected as a national-level young talent project; selected as a leading talent in science and technology innovation in Tianjin (2019), and an outstanding youth in Tianjin (2020).
Source of information: Energy Scholar
This information is from the Internet for academic exchanges. If there is any infringement, please contact us and delete it immediately